Cellular Metabolism
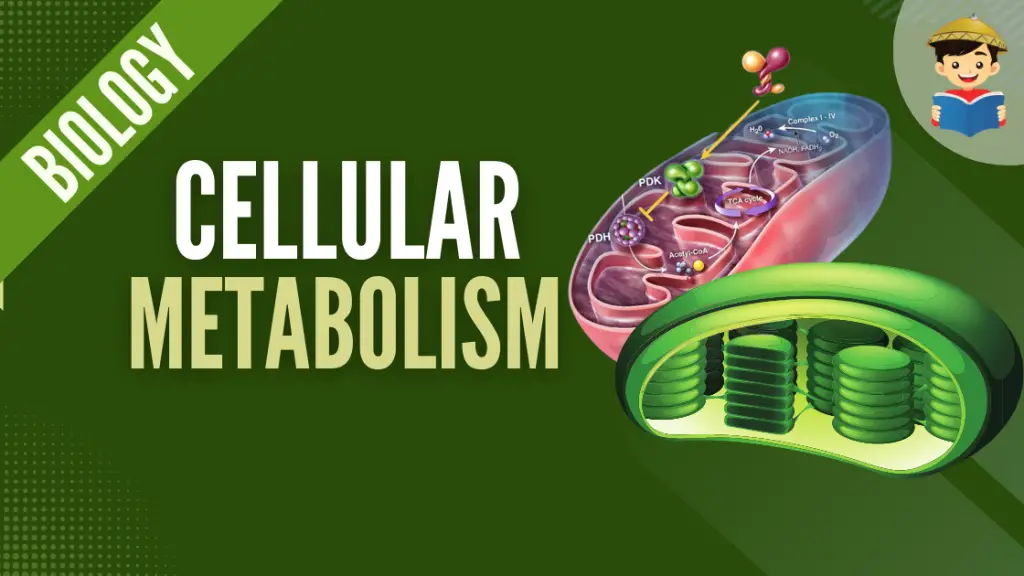
How does the food we eat become energy? Why are plants so important? In this reviewer, you will learn how cells are able to take substances from their environment and transform them into energy to fuel their bodies.
Click below to go to the main reviewers:
Table of Contents
- Introduction to Cellular Metabolism
- Part I. Cellular Respiration
- Part II. Photosynthesis
- References
- Download Article in PDF Format
- Test Yourself!
Introduction to Cellular Metabolism
Every living organism needs energy and some organisms have cells that allow them to create their food. These organisms are called autotrophs (plants doing photosynthesis is an example of an autotroph) but others need to eat and convert the food into energy. These are heterotrophs which also include us human beings.
Part I. Cellular Respiration
For many heterotrophs, energy is gained through the process of cellular respiration.
Cellular respiration is a catabolic process. It means larger compounds are broken down resulting in the release of energy. Imagine chewing food on a molecular scale.
Cellular respiration is simplified as follows:

On your left are the reactants and the products yielded are on the right. The chemical equation is the reverse of photosynthesis, and the equation simplifies the complex process which occurs in every cell. To better familiarize yourself with the entire process, we will dissect it into different steps.
Steps in Cellular Respiration
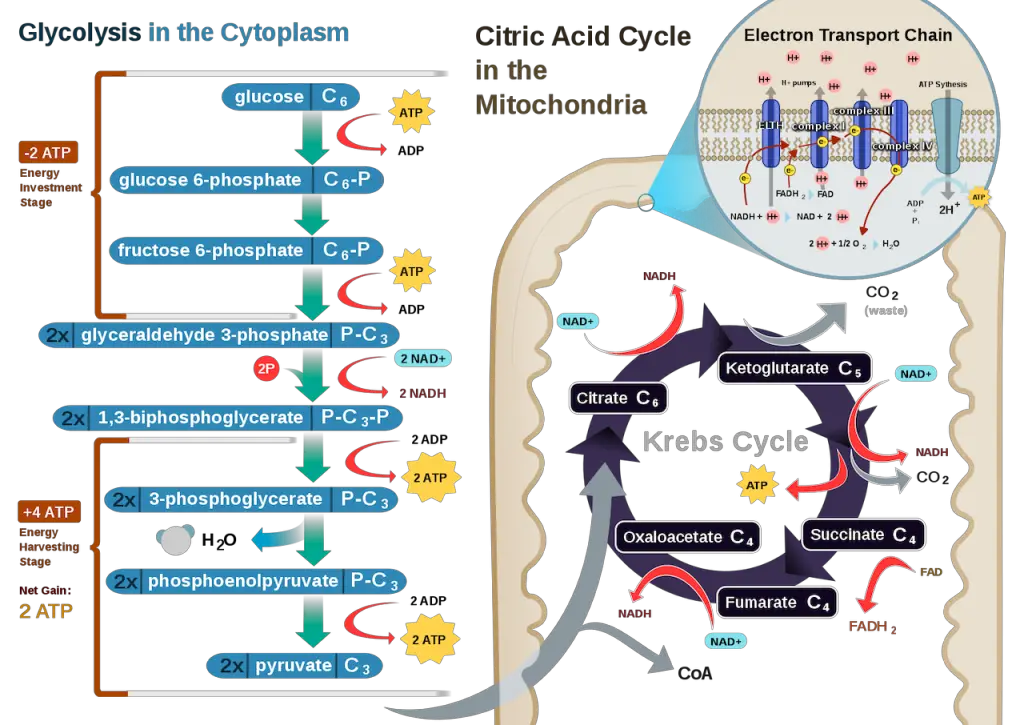
Cellular respiration can take place in the presence of oxygen (aerobic) or without it (anaerobic). For this portion, we will focus on aerobic respiration and mostly in the context of eukaryotes.
Life on Earth is based on carbon, and the food we eat is composed of a series of carbon molecules linked with hydrogen and other organic compounds.
Among the foods that are easier to digest and convert into energy are carbohydrates, compounds composed of carbon, hydrogen, and oxygen. The most abundant among them is the monosaccharide glucose (C6H1206). Energy is stored in the chemical bonds of these compounds and is released by breaking the links.
Before diving into the steps or stages, it is important to establish that the main goal of cellular respiration is to create energy that comes in the form of ATP (Adenosine Triphosphate). Think of it as a currency in the cell. In addition, proteins called enzymes, more specifically electron carriers, are essential in regulating and facilitating the process. Chief of this is nicotinamide adenine dinucleotide (NAD+/NADH) and then flavin adenine dinucleotide (FAD/FADH2).
Step 1: Glycolysis
The first step in cellular respiration is glycolysis, which simply means breaking up the glucose molecule.
The content of cells is suspended in the cytoplasm. Within this cytoplasm is a fluid called cytosol which also contains enzymes that aid the process. Once glucose enters this intracellular fluid, the enzymes break it into 2 pyruvic acid (or pyruvate, both used interchangeably) molecules.
This process requires 2 ATP, and as the step proceeds, it yields a net of 2 ATPs as there are two pyruvate molecules. In anaerobic cellular respiration, the process ends here when the glucose is split into 2 pyruvic acid molecules.
In addition, high-energy electrons are also transferred to the electron carrier NAD+ through reduction resulting in the electron carrier NADH with 2 NADH yielded in this step. These electron carriers are more important in the last step of cellular respiration.
Step 2: Pyruvate Oxidation

Animal mitochondrion diagram by LadyofHats is available in the public domain.
This is where the mitochondria and their importance in the process become apparent. The mitochondrion is a cellular unit called an organelle composed of two membranes, an inner and outer membrane.
The pyruvic acid is able to diffuse past these membranes and into the mitochondrial matrix, the innermost compartment of the mitochondrion. Pyruvic acid is split and then chained to an enzyme resulting in the 2-carbon compound Acetyl Coenzyme A (Acetyl CoA). The cleaved carbon combines with oxygen to form carbon dioxide, a waste product. Acetyl CoA is then subjected to the next process.
Step 3: Krebs Cycle, Citric Acid Cycle, or TCA (Tricarboxylic Acid) Cycle
Still in the matrix, the Krebs cycle is a closed loop; this means the last part of the cycle reforms the molecule used to initiate the step. The cycle involves eight major steps resulting in citrate, isocitrate, ketoglutarate, succinyl-CoA, succinate, fumarate, malate, and oxaloacetate.
A mnemonic I found useful in remembering the products of each step is “Citrate Is Kreb’s Starting Substrate For Making Oxaloacetate.” Each series of reactions release energy and is captured in molecules of NADH, ATP, and FADH2. Two turns will take place due to glycolysis producing two pyruvic acid molecules.
After the second turn of the cycle, the glucose molecule has been broken down completely with all six carbon atoms combined with oxygen to form carbon dioxide. This stage yields: 2 ATPs, 6 NADH, and 2 FADH2.
Step 4: Oxidative Phosphorylation
Oxidative phosphorylation is the last step in cellular respiration and has two stages: the electron transport chain and chemiosmosis.

“Electron Transport Chain. Image by Dw001. Licensed under CC BY-SA 4.0.
a. Electron Transport Chain (ETC)
The inner membrane of the mitochondria is filled with proteins called complexes (4 main complexes, labeled “complex I” to “IV,” in the mitochondrion) which move electrons from NADH and FADH2 to oxygen. Energy from the carriers is also transferred to ATP. An electron transport chain is a series of molecules that transfer electrons from one molecule to another by chemical reactions.
Some of the energy from the electrons pump the positively charged hydrogen ions (H+) from the matrix into the intermembrane space. NADH transfers electrons from complex I while FADH2 is only able to enter the chain starting at complex II. Thus, NADH yields more ATPs compared with FADH2.
b. Chemiosmosis
As more ions are pumped, this creates a difference in concentrations of charges, an electrochemical gradient, between the intermembrane space and the matrix. This gradient causes the ions to flow from high to low concentration, from the intermembrane space back into the matrix.
Facilitating this process is the protein ATP Synthase which acts as a channel, helping hydrogen ions cross the membrane. As its name suggests, it also forms ATP from ADP (Adenosine Diphosphate) by adding another phosphate. The flow of hydrogen ions through ATP synthase provides energy for ATP synthesis. After passing the electron transport chain, the electrons combine with oxygen and form water.
How Much Energy Do We Gain Through Cellular Respiration?
If you look at different references, you may notice that the amount of ATP produced by cellular respiration varies. Current sources estimate that the maximum ATP yield for one glucose molecule is around 30-32 ATP. This is because it accounts for the transport of ADP into the mitochondrion as well as taking ATP out of it.

For this article, we will follow current estimates that electron carrier NADH yields 2.5 ATPs while FADH2 yields 1.5 ATP during the electron transport chain. With this, we can do a little breakdown of one molecule of glucose:
Stage | Products | ATP Yield (in Oxidative Phosphorylation) |
Glycolysis | 2 ATP 2 NADH | 2 ATP 5 ATP |
Pyruvate Oxidation | 2 NADH | 5 ATP |
Krebs Cycle | 2 ATP 6 NADH 2 FADH2 | 2 ATP 15 ATP 3 ATP |
Total | 32 ATP |
Part II. Photosynthesis
Life on Earth is powered by the sun. Through the process of photosynthesis, plants use solar energy to convert carbon dioxide (CO2) and water (H2O) to sugars and other organic molecules, releasing oxygen (O2) as a by-product. In a way, photosynthesis is the reverse of cellular respiration and is considered an anabolic process, where complex and larger compounds are made from simpler building blocks.
All green plants have chloroplasts with the leaves being the major sites of photosynthesis. A leaf’s green color comes from chlorophyll, a light-absorbing pigment that plays a central role in converting solar energy to chemical energy.

If you examine a cross-section of a leaf, chloroplasts are concentrated within cells found in the mesophyll, the green tissue within leaves. CO2 and O2 enter or exit the leaf through tiny pores called stomata. Water absorbed by the plant is delivered to the leaves in veins. The veins are also used to export sugar to other parts of the plant.
In the chloroplast, two membranes enclose an inner compartment filled with a thick fluid called the stroma. Suspended in this fluid are interconnected sacs called thylakoids, which enclose another internal compartment, called the thylakoid space.
Thylakoids when arranged in stacks are called grana and built within the thylakoid membranes are the chlorophyll molecules that capture light energy. The thylakoid membranes also contain machinery that converts light to chemical energy, which is then used in the stroma of the chloroplast to make sugar.
The summary equation of photosynthesis, much like cellular respiration, is a simplification of linked processes, each with multiple steps.
Phase 1: Light-dependent Reaction
The first process occurs in the thylakoids. This light-dependent reaction or light reactions convert light to chemical energy and release oxygen. Water is split to provide electrons along with giving off oxygen as a result. Light energy is absorbed by chlorophyll molecules in the thylakoid membranes, and this is used to drive the transfer of electrons and H+ from water to the electron acceptor NADP+, reducing it to NADPH.
Think of NADPH as a cousin to cellular respiration’s NADH, only differing in NADPH having an extra phosphate group. NADPH temporarily stores electrons and provides “reducing power” to the next process. The light reactions also generate ATP from ADP and a phosphate group.
To summarize, the light reactions absorb light energy and convert it into chemical energy which is then stored in ATP and NADPH. But what is light in the context of a plant?
Sunlight and Photosynthetic Pigments
Light can act as either a particle or a wave. What concerns plants is that light is made of photons that contain a fixed amount of energy that they must harness. To do this, plants have different photosynthetic pigments that allow them to harness different wavelengths of sunlight. We do not see the absorbed wavelengths, we see those transmitted or reflected by the pigments, which in most cases are green.

Chloroplasts contain more than one pigment. Chlorophyll a is able to absorb mainly blue-violet and red light. A similar pigment, chlorophyll b, absorbs blue and orange light mainly. Chlorophyll b broadens the range of light plants can use by conveying their absorbed energy to chlorophyll a, which directly participates in the light reactions.
Chloroplasts also contain carotenoids which are the shades of yellow and orange we see in fall leaves when green chlorophyll breaks down, and carotenoids show through; and in other plants, we perceive them as those colors. They can function in photosynthesis, but they are more for protection since carotenoids seem to absorb and dissipate excess light energy that would otherwise damage chlorophyll. They also react with oxygen, which can damage cell molecules due to their reactivity.
So how does a pigment absorb light? And what do plants do with it?
In the thylakoid membrane, chlorophyll molecules are organized into clusters called photosystems. A photosystem contains two complexes: a reaction-center complex surrounded by many light-harvesting complexes. The light-harvesting complex contains many pigment molecules bound to proteins; the number and variety of pigments can harvest more light over a large surface area, and a wider range of the light spectrum than a single pigment absorbs alone.
Together, these light-harvesting complexes act in gathering energy that is transferred from one molecule to another. Think of a human “wave” in sporting events. Energy is transferred until it reaches the reaction-center complex which contains a pair of special chlorophyll a molecule, called a primary electron acceptor, capable of accepting electrons and becoming reduced.
When an electron from a reaction-center chlorophyll a is boosted to a higher energy level (this state is also called an “excited” state), it is immediately captured by the primary electron acceptor. This is the first step in transforming light into chemical energy in light reactions.

Light-dependent reactions of photosynthesis in the thylakoid membrane of plant cells. Image by Somepics. Licensed under CC BY-SA 4.0
Two types of photosystems have been identified which cooperate in the light reactions. They are referred to as photosystems I and II, in order of their discovery. In the reaction, however, photosystem II acts first in the sequence that makes up the light reactions.
Each of the two photosystems has a distinct reaction-center complex, which contains a special pair of chlorophyll a molecules that are named according to the maximum wavelength of light they can absorb: P700 is the name of the chlorophyll a in photosystem I while those of photosystem II is called P680.
Now, we will look at how these photosystems work together in light reactions to generate ATP and NADPH.
Electron Transport Chain in Plants
How do the captured electrons lead to the production of ATP and NADPH?
Part of it is the arrangement of the photosystems in the thylakoid membranes and their connection through an electron transport chain. This arrangement is often called the Z scheme. Another is the flow of electrons removed from water through these components to NADPH. The final part is the synthesis of ATP which is linked to an ETC that pumps H+ into a membrane compartment which flows through ATP synthase embedded in the membrane, much like in cellular respiration.
You might have seen illustrations of the ETC in plants such as the following:
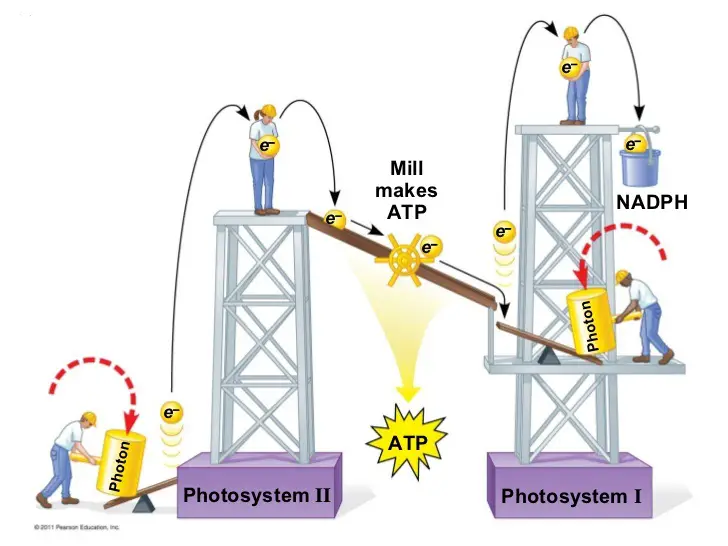
But this simple analogy leaves a few questions unanswered: Where do the electrons that move through the photosystems to NADPH come from? And we know the light reactions produce oxygen, so where does this happen? And how does the flow of electrons down ATP synthase produce ATP?
We will slowly answer those questions starting with the first one.
The electrons that reduce NADP+ to NADPH come from water. The thylakoid space has enzymes that split water into two electrons, two hydrogen ions, and an oxygen atom. The oxygen atom immediately joins another oxygen atom forming oxygen gas (O2). Water is the source of O2 produced in photosynthesis, and these oxygen molecules diffuse out the thylakoids, the chloroplast, and the plant cell, finally exiting the leaf through the stomata.
The electrons from water are passed, one by one, to the chlorophyll a in photosystem II, replacing the excited electron that was captured by the primary electron acceptor. From photosystem II, the electrons pass through the ETC to the reaction center chlorophyll a in photosystem I, again replacing the excited electron that has been captured by its primary electron acceptor. The electrons are passed through a short electron transport chain to NADP+, reducing it to NADPH.
All that is left now is ATP. It’s simpler, the players and processes we met in ATP synthesis in cellular respiration play the same role in plants. Recall that in chemiosmosis, the concentration gradient from the pumping of H+ powers ATP synthesis with the rotating ATP synthase phosphorylating ADP to produce ATP.
We pretty much tackled everything there is about light-dependent reactions, but photosynthesis is also made up of another process.
Phase 2: Light-independent Reactions
The light-independent reaction is also known as the Calvin cycle. It occurs in the stroma of the chloroplast. It is a series of reactions that assemble sugar molecules using carbon dioxide and the energy-rich products of the light reactions. The incorporation of carbon from carbon dioxide into organic compounds is called carbon fixation. After fixing carbon, the carbon compounds are reduced to sugars.
The NADPH from the light reactions provides electrons that reduce carbon compounds in the Calvin cycle while the ATP from the light reactions provides the chemical energy that fuels several steps of the Calvin cycle. Although the Calvin cycle is referred to as the dark reactions or light-independent reactions, in most plants the cycle occurs during the day when the light reaction is able to supply the cycle with NADPH and ATP.
We will now take a closer look at the different steps in the Calvin Cycle.
The Process of Making Sugar: A Closer Look at the Calvin Cycle
The Calvin cycle is like a sugar factory in chloroplasts. ATP and NADPH from the light reactions are used to reduce carbon dioxide into sugars. The output of this cycle is an energy-rich three-carbon sugar, glyceraldehyde-3-phosphate (G3P). Plants make use of this G3P to form glucose, sucrose, and other organic molecules as needed.

The Cycle can be described in four main steps with the starting material being a five-carbon sugar called ribulose biphosphate (RuBP). To make a molecule of G3P, the cycle must turn thrice, incorporating three molecules of CO2 molecules to end up with a complete G3P molecule.
The four main steps can be described as follows:
- Carbon Fixation occurs when the enzyme rubisco attaches CO2 to RuBP. This unstable six-carbon molecule splits into two three-carbon molecules.
- Reduction occurs after, where ATP and NADPH are used to reduce the three-carbon molecule to G3P.
- The next step releases one molecule of G3P, this occurs every three CO2 molecules that are fixed.
- The last step regenerates RuBP. It is a series of chemical reactions which uses ATP to rearrange the atoms in five G3P molecules to form three RuBP molecules.
For synthesizing one G3P molecule, the Calvin Cycle consumes 9 ATP and 6 NADPH molecules, which were provided by the light reactions. Neither light reactions nor the Calvin cycle alone can make sugar from carbon dioxide, so photosynthesis is an integration of these two processes that emerged from the organization of structures in the chloroplast.
This is how photosynthesis occurs in most plants, but because plants are diverse and must adapt to certain environments, some plants make use of specialized modes of photosynthesis.
Adapted to Dryness: C4 and CAM Plants
Most plants use CO2 directly from the air, and carbon fixation occurs when rubisco adds CO2 to RuBP, resulting in a stable three-carbon intermediate compound. Such plants are called C3 plants because of this. However, in hot, dry weather, C3 plants tend to close their stomata which prevents water loss but also prevents CO2 from entering the leaf, and this slows photosynthesis. The O2 from the light reactions also accumulates, which creates another problem.
As O2 builds up in the leaf, rubisco adds the O2 instead of CO2 to RuBP. The two-carbon product of this reaction is broken down in the cell in a process called photorespiration, but unlike cellular respiration, it uses ATP instead of creating it, and unlike photosynthesis, it yields no sugar.
Some hypothesized this to be a relic from a time when the atmosphere had less oxygen than it does today, while others noted evidence that this role of rubisco helps protect the plants from the accumulation of products of the light reactions in the cell.
Two adaptations were made by some plants to be able to deal with dry conditions.

The first one is exhibited by plants in hot, dry climates where there are alternate modes of carbon fixation that minimize photorespiration and optimize the Calvin cycle. These plants, called C4 plants, are named because they fix CO2 into a four-carbon compound. During hot or dry weather, the plant keeps its stomata mostly closed to conserve water.
It continues making sugars by photosynthesis where it fixes carbon in mesophyll cells and proceeds with the Calvin cycle in the bundle sheath cells. An enzyme in the mesophyll cells has a high affinity for CO2 and can fix carbon even if carbon dioxide concentrations in the leaf are low. The resulting four-carbon compound acts as a CO2 shuttle as it moves into bundle sheath cells, which are packed around the veins of the leaf, and releases CO2.
Thus, the CO2 concentrations in these cells remain high enough for the Calvin cycle to continue making sugar instead of photorespiration. Corn and sugarcane are examples of important C4 plants we grow in agriculture.
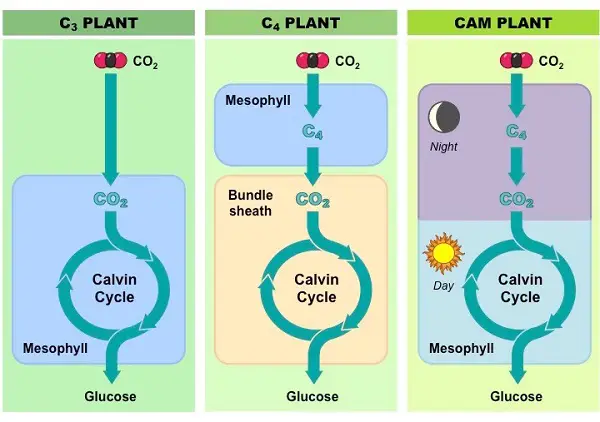
Another adaptation is exhibited by pineapples, many cacti, and other succulent plants. Referred to as CAM (Crassulacean Acid Metabolism) plants they are well-adapted to very dry climates. The acronym for CAM refers to the plant group where the phenomenon was first examined, which belongs to the succulents known as the Crassulaceae.
CAM plants conserve water by opening their stomata and allowing CO2 to enter only at night. CO2 is fixed as a four-carbon compound, which stores CO2 at night and releases it during the day. This allows the Calvin cycle to operate even if the stomata close during the day.
In C4 plants, carbon fixation and the Calvin cycle occur in different cells while in CAM plants, these processes occur in the same cells, but at different times of the day. Keep in mind, however, that CAM, C4, and C3 plants eventually make use of the Calvin cycle to make sugars from CO2.
References
Alberts, B., Johnson, A., Lewis, J., Raff, M. C., Roberts, K., Walter, P., … Hunt, T. (2008). Molecular Biology of The Cell. New York: Garland Science.
Armstrong, P. (2007). All things Darwin: An encyclopedia of Darwin’s world. Westport, Connecticut: Greenwood.
Campbell, N. A., Cain, M. L., Minorsky, P. V., Reece, J. B., Urry, L. A., & Wasserman, S. A. (2018). Biology: A global approach. Harlow, Essex, England: Pearson Education Limited.
Chiras, D. D. (2008). Human Biology. Sudbury, Mass: Jones and Bartlett Publishers.
Fowler, S., Roush, R., & Wise, J. (2013). Concepts of Biology. Houston, Texas: OpenStax. Available at https://openstax.org/books/concepts-biology/pages/1-introduction.
Hillis, D. M., Moritz, C., & Mable, B. K. (1996). Molecular systematics. Sunderland, Mass: Sinauer.
Hine, R. (2019). A Dictionary of Biology. Oxford, US: Oxford University Press.
Hollingsworth, P. M., Bateman, R. M., Gornall, R. J., & Conference on Advances in Plant Molecular Systematics. (1999). Molecular systematics and plant evolution. New York: Taylor & Francis.
Jamora, C., Fuchs, E. (2002). Intercellular adhesion, signaling, and the cytoskeleton. In: Nature Cell Biology. Volume 4, E101–E108. Available at https://doi.org/10.1038/ncb0402-e101
Karp, G., Iwasa, J., & Marshall, W. (2018). Cell biology. Hoboken, NJ: John Wiley.
Lecuit, T., Pilot, F. (2003). Developmental control of cell morphogenesis: a focus on membrane growth. In: Nature Cell Biology. Volume 5, pages 103–108. Available at https://doi.org/10.1038/ncb0203-103
Lodish, H. F. (2013). Molecular Cell Biology. New York: W.H. Freeman and Co.
Meerman, R., & Brown, A. (2014). When somebody loses weight, where does the fat go? British Medical Journal. Vol. 2014. Issue 349.
Nelson, D. L., & Cox, M. M. (2017). Lehninger Principles of Biochemistry (7th ed.). W.H. Freeman.
Ochman, H. (2016). Microbial evolution: A subject collection from Cold Spring Harbor Perspectives in Biology. Cold Spring Harbor, New York: Cold Spring Harbor Laboratory Press.
Reece, J. B., & Campbell, N. A. (2011). Campbell Biology. Boston: Benjamin Cummings/Pearson.
Simpson, M. G. (2019). Plant systematics. Amsterdam Academic Press.
Taylor, M. R., Simon, E. J., Dickey, J., Hogan, K. A., & Reece, J. B. (2018). Campbell Biology: Concepts & connections. NY: Pearson.
Next topic: The Central Dogma of Biology
Previous topic: The Cell
Return to the main article: The Ultimate Biology Reviewer
Download Article in PDF Format
Test Yourself!
1. Practice Questions [PDF Download]
2. Answer Key [PDF Download]
Written by Earl Jeroh Bacabac
in College Entrance Exam, LET, NMAT, Reviewers, UPCAT
Earl Jeroh Bacabac
Earl’s love for the sea fueled his goal to become a marine biologist. He obtained his Bachelor’s Degree in Biology from the University of the Philippines Visayas while also being a DOST scholar. His passion for the marine environment is rivaled by his diverse interests in music, the arts, and video games.
Copyright Notice
All materials contained on this site are protected by the Republic of the Philippines copyright law and may not be reproduced, distributed, transmitted, displayed, published, or broadcast without the prior written permission of filipiknow.net or in the case of third party materials, the owner of that content. You may not alter or remove any trademark, copyright, or other notice from copies of the content. Be warned that we have already reported and helped terminate several websites and YouTube channels for blatantly stealing our content. If you wish to use filipiknow.net content for commercial purposes, such as for content syndication, etc., please contact us at legal(at)filipiknow(dot)net